Processing Methods for the Preparation of Porous Ceramics
Article information
Abstract
Macroporous ceramics with tailored pore size and shape could be used for well-established and emerging applications, such as molten metal filtration, biomaterial, catalysis, thermal insulation, hot gas filtration and diesel particulate filters. In these applications, unique properties of porous materials were required which could be achieved through the incorporation of macro-pores into ceramics. In this article, we reviewed the main processing techniques which can be used for the fabrication of macroporous ceramics with tailored microstructure. Partial sintering, replica templates, sacrificial fugutives, and direct foaming techniques was described here and compared in terms of micro-structures and mechanical properties that could be achieved. The main focus was given to the direct foaming technique which was simple and versatile approach that allowed the fabrication of macro-porous ceramics with tailored features and properties.
1. Introduction
Porous ceramics are essential engineering materials due to their high level of demand in several applications depending upon the pore size. During the last decade, tremendous efforts have been devoted for the researches on innovative processing technologies of porous ceramics, resulting in better control of the porous structures and substantial improvements of the properties [1, 2]. This is mainly associated with the properties such as high surface area, high permeability, high porosity, low specific heat and high thermal insulation. According to the nomenclature of IUPAC (International Union of Pure and Applied Chemistry), porous materials are classified into three grades depending on the pore diameter d: macroporous (d > 50 nm), meso-porous (50 nm > d > 2 nm) and microporous (d < 2 nm) [3].
For structural applications of brittle ceramic materials, pores are generally want to be eliminated because they act as fracture defects and degrade the structural reliability, and therefore, ceramic engineers tried to sinter ceramics to full density to attain high mechanical strength. On the other hand, there have been various industrial applications where pores are taken advantage of positively such as, high temperature filtration [4], orthopedic implants [5], thermal insulation [6], catalyst supports [7], lightweight structural components and diesel particulate filters [8]. In the past ten years, a great deal of research efforts have been devoted for tailoring deliberately sizes, amounts, shapes, locations and connectivity of distributed pores, which have brought improved or unique properties and functions of porous ceramics [9-18]. Different processing techniques creates pore of various size ranges as shown in Fig. 1. A tremendous amount of research works reported in this field these days on manufacturing porous ceramics using different techniques. This review mainly focuses on the recent results obtained in the preparation of macroporous ceramics utilizing the particle-stabilized direct foaming technique. The aim of this article is to compare the currently available processing routes for the preparation of macroporous ceramics especially particle-stabilized direct foaming technique. We also present recent important results by the authors that has not been included in the previous reviews.
2. Processing Methods for Macroporous Ceramics
The processes for manufacturing macroporous ceramics can be divided into following four categories and the process schematic is shown in Fig. 2.
Partial sintering
Replica templates
Sacrificial fugitives
Direct foaming
2.1. Partial sintering
Partial sintering of powder compact is the most conventional and straightforward route to fabricate porous ceramic materials. In this technique, particles of powder compact are bonded due to surface diffusion or evaporation- condensation processes enhanced by heat treatments. A homogeneous porous structure forms when sintering is terminated before fully densification occur. Pore size is controlled by the size of starting powders whereas porosity is controlled by the degree of partial sintering.
Generally, in order to provide the desired pore size, the size of raw powder should be geometrically in the range two to five times larger than that of pore. The mechanical properties depend largely on degree of neck growth between grains, as well as porosity and pore size. Several processing approaches have been developed to enhance grain bonding and improve strength of porous ceramics. Oh et al. [19] and Yang et al. [20] fabricated porous Al2O3 and Al2O3 based composites by the pulse electric current sintering (PECS) technique and found that the strength was substantially improved due to the formation of thick and strong necks. Deng et al. [21, 22] tried to obtain strong grain bonding through combination of partial sintering and powder decomposition. Al(OH)3 experiences a 60% volume contraction during decomposition and produces fine Al2O3 grains. The fracture strength of obtained porous Al2O3 higher than that of the pure Al2O3 sintered specimens because of strong grain bonding that resulted from the fine Al2O3 grains produced by the decomposition of Al(OH)3.
2.2. Replica templates
Macroporous ceramics having interconnected large pores of high volume porosity and open cell walls have been frequently fabricated by the replica techniques. The first step of a typical template process is impregnation of a porous or cellular structure with ceramic suspension or precursor solution. The most frequently used synthetic template is porous polymeric sponge such as polyurethane. Natural resources of porous structures such as woods, corals, sea sponge, etc. have been also used as replica templates. The templates are soaked into a ceramic slurry or precursor solution to impregnate them, and the surplus is drained and removed by centrifugation or roller compression. In this process, the appropriate viscosity and fluidity depending on the cell size are required so that uniform ceramic layer forms over the sponge walls. The ceramic-impregnated templates are dried and then heat treated to decompose the organic sponges. Following the pyrolysis, the ceramic layers are sintered at higher temperatures for densification. Porosity higher than 90% can be obtained with cell sizes ranging from a few hundred micrometers to several millimeters. The open cells are interconnected, which allows fluid to pass through the foams with a relatively low pressure drop. However, due to cracking of the struts during the pyrolysis, the mechanical properties of ceramic reticulated foams are generally poor [23]. In order to avoid the strut crack formation, a variety of approaches have been developed. In order to increase the struts thickness and heal the strut cracks, Zhu et al. [24] recoated repeatedly a reticulate porous ceramic body with thinner slurry of the same composition; the green body coated with thinner slurry is then preheated to burn out the sponge followed by sintering.
2.3. Sacrificial fugitives
Porous ceramics can be obtained by mixing appropriate amounts of sacrificial fugitives as pore forming agents with ceramic raw powder and evaporating or burning out them before or during sintering to create pores [25]. The pore forming agents are generally classified as follows:
Synthetic organic matters such as polymer beads, organic fibers.
Natural organic matters such as potato starch, cellulose, cotton.
Metallic and inorganic matters such as nickel, carbon, fly ash, glass particles.
Liquid such as water, gel, emulsions.
Porosity is controlled by the amount of the agents, and pore shape and size are also affected by the shape and size of the agents respectively when their sizes are large in comparison with those of starting powders or matrix grains. This approach is useful particularly for obtaining high open porosity. The agents, however, need to be mixed with ceramic raw powder homogeneously for obtaining uniform and regular distribution of pores. Polymethylmethacrylate (PMMA) beads and microbeads have been frequently employed for sacrificial fugitives [26-28]. Cruz et al. [29] used a colloidal processing technique with PMMA sacrificial templates, to fabricate macroporous yttria-stabilized zirconia ceramics. Song et al. [30] produced micro-cellular silicon carbide ceramics with a duplex pore structure by using expandable micro-spheres and PMMA spheres; which resulted in the large pores and the small windows in the strut area respectively. However, the removal of the organic template material can be very time consuming and generates harmful byproducts.
2.4. Direct foaming
In this method, porous materials are fabricated by foaming the ceramic slurry by mechanical agitation or in situ evolution of gases followed by drying and consolidation to obtain high-strength porous materials [1, 2]. This approach probably yields the widest range of cellular structures and hence properties, but they are generally less open than the replicated foams. This technique allows low cost and easy production of highly porous ceramic materials, up to more than 95% porosity. The total porosity of directly foamed ceramics is proportional to the amount of gas incorporated into the suspension or liquid medium during the foaming process.
Liquid foams are thermodynamically unstable systems due to their high gas-liquid interfacial area. Several physical processes take place in wet foams to reduce the total Gibbs free energy of the system, resulting in large pores in the final porous bodies, leading to foam destabilization. The main destabilization mechanisms are drainage (creaming), coalescence (film rupture), Ostwald ripening (disproportionation), and sedimentation as shown in Fig. 3. The combined action of all these physical processes may collapse the foam within a few seconds after air incorporation. The foam lifetime can be increased to a few minutes or several hours by adsorbing long-chain surfactants. Remarkably stable foams exhibiting lifetime of several days and weeks can be prepared through the adsorption of colloidal particles on the surface of air bubbles.
3. Direct Foamimg for Macroporous Ceramics
Following two approaches can be used in order to stabilize the wet foams.
Surfactant-stabilized foams
Particle-stabilized foams
3.1. Surfactant-stabilized foams
One of the most frequently approaches for the stabilization is to use surfactants which reduce the interfacial energy of the gas/liquid boundaries. Several long chain surfactants are used for the stabilization and they are classified into several types including non-ionic, anionic, cationic and proteins as depicted in Table 1. By controlling the foam stability and the setting kinetics, pore sizes within the range of 35 μm to 1.2 mm have been achieved using the surfactant-based direct foaming method [2], depending on how effectively the used surfactants work.1
These surfactant molecules slow down the coalescence and disproportionation of bubbles by adsorbing at the air bubble surface and reducing the air/water interfacial energy. However, long chain surfactants and biomolecules have low adsorption energy at the air/water interface; therefore they cannot prevent the long-term destabilization of foams. Wet foams stabilized with long-chain surfactants collapse within a few minutes after foaming, whereas those stabilized by proteins exhibit bubble disproportionation within a few hours. Therefore, a setting agent is required to consolidate the foam microstructure before extensive coalescence and disproportionation take place. A schematic showing the stabilization with surfactant is shown in Fig. 4. The porosity of cellular structures produced via surfactant-based direct foaming can be tuned from approximately 40% up to 97%. The pores obtained with this method are typically spherical and can be either closed or opened depending on the foam wet processing. Open pores exhibiting interconnecting windows are obtained if particles segregate at the plateau borders of the foam because of bubble disproportionation. Closed pores, on the other hand, are typically achieved when the particles are distributed uniformly around the gas bubbles upon setting. These conditions can be controlled in the process by adjusting the foam stability, air content, particle concentration, and setting kinetics.
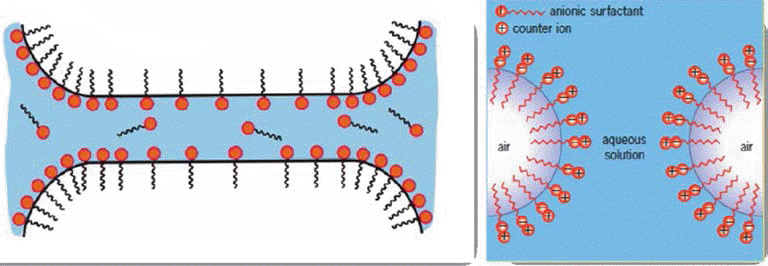
Stabilization of air/water interface through the adsorption of amphiphile molecule at the interface.
Santos et al. [31, 32] applied the sol-gel technique to produce ZrO2 porous ceramics from the condensation reaction of zirconium oxychloride in combination with Freon and surfactants. He et al. [33] manufactured 3D interconnected zirconia toughened alumina foam using egg white protein as a binder and foaming agent for potential bone graft applications. As opposed to the replica techniques, the direct foaming methods usually lead to dense flawless struts after sintering, which markedly increase the mechanical strength of the porous ceramic in comparison with the replica derived, structures. For porosities higher than 90% the cell walls are markedly thin, leading to lower mechanical strength than that theoretically estimated for open-cell structures. However, by decreasing porosity the pores gradually change from a highly open to a completely closed morphology, increasing the strength towards the level predicted for closedcell structures.
3.2. Particle-stabilized foams
The equilibrium contact angle (θ) is determined by a balance between the interfacial tensions involved. Foams are obtained for contact angles lower than 90°, whereas mists (aerosols) are produced for contact angles varying from 90 to 180° as shown in Fig. 5 [1].
The basic requirement for the preparation of materials utilizing particle-stabilized direct foaming technique is the use of colloidal particles that strongly adsorb at air/ water interfaces. This adsorption at interface occurs when particles are not completely wetted by any of the fluids, thus exhibiting a finite equilibrium contact angle at the triple phase boundary. The contact angle of colloidal particles at fluid interfaces depends on the surface chemistry, roughness, impurities, particle size, as well as on the composition of the fluid phases. Different materials have different contact angles at the solid/liquid/gas interface as shown in Fig. 6. Ceramic materials exhibit very high surface free energies and therefore are in principle fully wettable. However, the wettability of the particles can be tuned by the adsorption of amphiphile on their surfaces to render them partially hydrophobic. Controlling the contact angle of particles at the interface is of major importance when applying this processing route, since it determines the type and stability of the initial particle-stabilized foams and emulsions. Moreover, tailoring the contact angle of particles with different chemical compositions should enable the preparation of foams and emulsions with a variety of new functional materials. The particle behavior in the liquid depending upon the contact angle with the interface is shown in Fig. 7.
Gonzenbach et al. [34-37] developed particle-stabilized direct foaming technique in order to obtained macroporous ceramics and demonstrated that the foams are stable for several days compared with ordinary surfactant stabilized foams which collapse in the first hour. In this method, the attachment of colloidal particles at the air/water interface is promoted by deliberately changing the wettability of the particle upon adsorption of shortchain amphiphilic molecules on the surface. The amphiphiles initially added to the suspension render the particle partially hydrophobic by adsorbing on their surface. The attachment of colloidal particles at the air/water interface is promoted by adjusting the wettability of the particle upon adsorption of short-chain amphiphilic molecules on the surface. Because of its remarkable stability, the particle- stabilized foams can be dried directly in air without crack formation. The macroporous ceramics obtained after sintering exhibit porosities from 45 to 95% and cell sizes between 10 μm and 300 μm. The surface-modified particles which originally cover the air bubble in wet foams become a thin surface layer of single grains after sintering. Macroporous ceramics with open porosity can be also prepared with this technique by simply decreasing the concentration of stabilizing particles. Processing steps in the particle-stabilized direct foaming technique are shown in Fig. 8.
Ahmad et al. [38] studied the foamability and foam stability of zirconia foam using valeric acid as an amphiphile as shown in Fig. 9. It is noteworthy that the zirconia foam showed good foamability and stability with a valeric acid concentration of 10 mmol/L and a uniform cell size was achieved ranging from 50 to 150 μm with porosity that exceeded 90%. The zirconia foam with tailored microstructure was successfully prepared and effect of amphiphile concentration on the particle agglomeration and sintering temperature on the strut wall thickness was also elaborated.
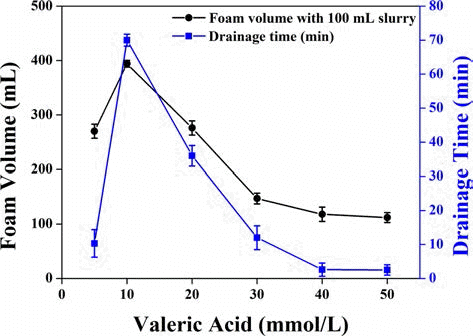
Foam volume and drainage time of zirconia foam prepared with 35 vol.% solid loading as a function of valeric acid concentration.
Ahmad et al. [39] successfully prepared ultra-low density zirconia toughened alumina (ZTA) foam with a uniform distribution of ZrO2 in the Al2O3 matrix and with single strut walls as shown in Fig. 10. ZTA foam has porosity of about 90% with compressive strength up to 8 MPa and has a cell size ranging from 80 μm to 200 μm.
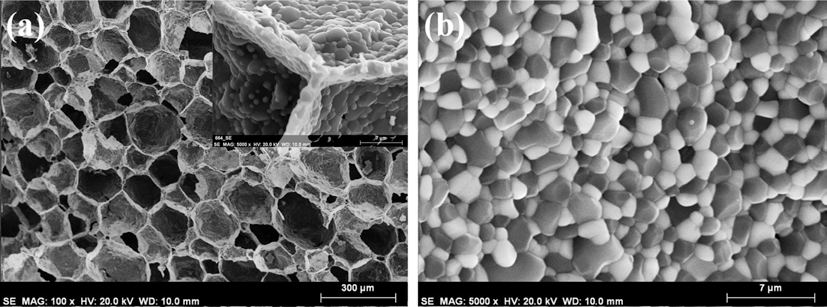
Particle-stabilized ZTA foam Al2O3-30 vol.% ZrO2 sintered at 1700 °C (a) showing cells and single strut wall in the inset, and (b) details of strut wall showing uniform distribution of ZrO2 in Al2O3 matrix.
Ha et al. [40] manufactured particle-stabilized diatomite foam with a bimodal pore structure that have primary pores with a size of 50 to 200 μm formed by the particles irreversibly adsorbed at air/water interface and secondary pores with a size of about 2 to 5 μm. They suggested that irregular and porous diatomite particles could be foamed on condition that the particle size was below than 10 μm. The air permeation properties of the diatomite foam were proportional to the sintering temperature up to 1200°C, which was enhanced by primary pores, and could increase filtration potential. The air permeation properties of the diatomite specimens prepared by sacrificial template method and particle-stabilized direct foaming method is shown in Fig. 11.
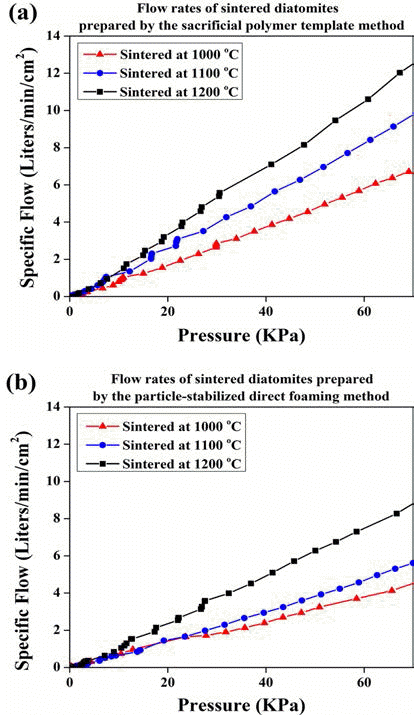
The air permeation properties of the diatomite specimens sintered at 1000 to 1200°C, prepared: (a) by the sacrificial polymer template method; and (b) by the particle-stabilized direct foaming method.
Ahmad et al. [41] showed that closed cell alumina foam can be manufactured by particle-stabilized direct foaming technique and it is possible to tailor the finally sintered Al2O3 foam properties by varying the amount of valeric acid, solid loading and stirring speed. The porosity of that alumina foam can be varied from 75 to 93 %, and cell size of the foam was between 20 to 300 μm. Particle-stabilized foams have inherently closed cell structure, but the open cell foams having can be formed by adjusting the solid loading. Ahmad et al. [42] introduced a new idea of preparation of open cell alumina foam using Al(OH)3 as a starting material utilizing direct foaming technique. In this process, the macropores were created by the foaming process and when sintering of this material was performed, the Al(OH)3 transformed into alumina, thus creating another porosity in the strut wall porosity without using any templates as shown in Fig. 12. Therefore, a material with open duplex pore structure can also be prepared using particle-stabilized direct foaming technique.
Ahmad et al. [43] enhanced the compressive strength of the ultra-high porous alumina foam manufactured by particle-stabilized direct foaming technique. AKP-30 and ALM-44 coatings with thicknesses of 50 μm and 140 μm, respectively, were successfully fabricated on highly porous substrates with a cell size ranging from 70 to 200 ìm and a porosity of 90% utilizing a simple dip-coating process. After sintering, the coatings exhibited good adhesion to a porous substrate without delamination. The increase in the compressive strength of porous Al2O3 substrates afforded by the coatings was believed to be due to the healing of cracks and surface defects. These materials can be useful in low-weight structural applications requiring a clean environment without debris along with good compressive strength. The fractured surface of the AKP-30 coating is shown in Fig. 13.
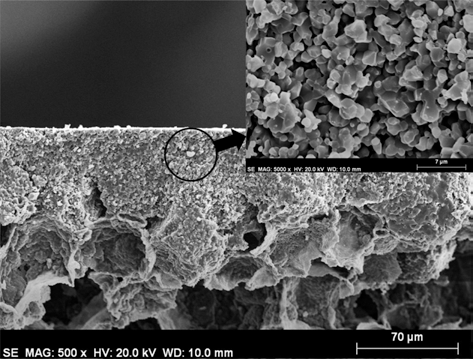
SEM image of the cross-section AKP-30 coating showing a uniform coating layer on a highly porous substrate with details of coating in the inset sintered at 1600 °C for 1 h.
Ha et al. [44] successfully prepared self-setting particle- stabilized zeolite 13X foams which can be commonly used as absorbent for wide range of industrial applications. These foams contain primary macropores formed by zeolite 13X particles that were irreversibly adsorbed at air/water interfaces as well as secondary inherent micropores. In addition, the permeability can be enhanced to the level of the typical ceramic membranes due to the highly interconnected primary macropores. The compressive strength and permeability of the self-setting particle-stabilized zeolite 13X foam with/without lithium carbonate and the sintered particle-stabilized zirconia foam is shown in Fig. 14.
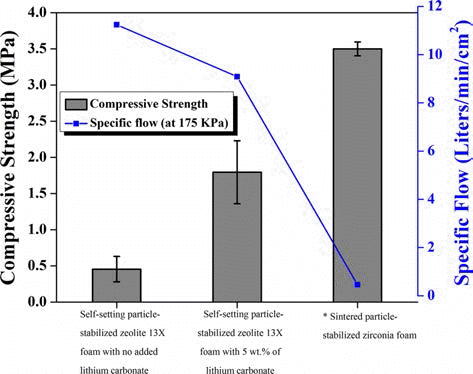
The compressive strength and permeability of the self-setting particle-stabilized zeolite 13X foam with/without lithium carbonate and the sintered particle-stabilized zirconia foam.
Chuanuwatanakul et al. [45] studied the microstructure (amount of porosity, average pore size, and morphology) of alumina foam as a function of surfactant (1-butane sulfonate (C4), 1-heptane sulfonate (C7), 1-decane sulfonate (C10)) concentration and chain length in combination with gel casting. Liu et al. [46] described the processing and properties of particle-stabilized porous lead zirconate titanate (PZT) ceramics using short chain amphiphile (valeric acid) followed by gel casting. Yu et al. [47] prepared the silicon nitride ceramic foams with nest like cell structure by particle- stabilized direct foaming technique and compared the foam stability with long and short chain surfactant molecules. Tseng et al. [48] manufactured gibbsite foams from particle stabilized emulsions using sodium dodecyl sulfate as a surfactant and corn starch as a binder. Lo et al. [49] prepared alumina foams having low thermal conductivity for SOFC insulations utilizing particle-stabilized direct foaming technique using SDS and valeric acid as a surfactant. Wong et al. [50] fabricated macroporous ceramic/ polymer composite foams by particle-stabilized technique and modification of interfacial energies was accomplished through surface modification of particles or by decreasing the surface tension of the aqueous phase. Therefore particle- stabilized direct foaming is a simple, cheap, and environmentally- friendly method for the fabricated porous ceramics of various porosity levels and pore size ranges with many different chemical compositions.
4. Summary
In this review paper, a number of approaches are discussed for the preparation of macroporous ceramics along with their advantages and disadvantages. In the past ten years, a lot of efforts have been devoted on the processing of porous ceramics in order to control the pore size, pore shape along with substantial improvements of properties. This review is mainly focused on the recent developments in the processing of macro-porous ceramics along with some important results obtained especially in particle-stabilized direct foaming technique. The techniques for the preparation of macroporous ceramics can be divided into four categories such as (i) partial sintering, (ii) replica templates, (iii) sacrificial fugitives, and (iv) direct foaming. The most conventional route for the preparation of porous ceramics is partial sintering in which the mechanical properties depend largely on degree of neck growth between grains, as well as porosity and pore size. Replica techniques produce the macroporous ceramics having interconnected large pores of high volume porosity and open cell walls but due to cracking of the struts during the pyrolysis, the mechanical properties of ceramic foams are poor. Sacrificial fugitives can be used as pore forming agents with ceramic raw powder which upon evaporating or burning create pores. In this technique, porosity is controlled by the amount of the agents, and pore shape and size are also affected by the shape and size of the agents. However, the removal of the organic template material can be very time consuming and generates harmful byproducts.
Direct foaming is a unique and versatile technique for the manufacturing of macro-porous ceramics. Surfactants and particles both stabilize interfaces by lowering the total energy of the system. Ceramic foams stabilized by particles are more resistant to disproportionation and drainage than the foam stabilized by surfactants. In fact particle-stabilized ceramic foams are so stable cellular microstructures which survive removal of the liquid phases during drying. By controlling the foam stability and the setting kinetics, pore sizes within the range of 35 μm to 1.2 mm can be achieved. The porosity of cellular structures produced via direct foaming can be tuned from approximately 40 to 97%. Using this simple, cheap, and environmentally-friendly method, porous ceramics of various porosity levels and pore size having different chemical compositions can be fabricated. In general, macroporous ceramics prepared by particle-stabilized direct foaming technique are impurity free, closed or open pore structure, high air contents, and isotropic cells. These advantages of using particle-stabilized direct foaming technique over conventional techniques to produce macroporous ceramics are obvious. The microstructure of ceramic foam can be tailored according to the required application by varying different parameters. These ceramic foams with open or closed cell structure can be used in a wide range of applications such as high temperature filtration, biomaterial, thermal insulations, and support for catalytic reactions.
Finally it can be concluded that porous ceramics are very important materials which can be used in a wide range of structural and functional applications. Great efforts are required for ensuring the good mechanical strength of highly porous materials. Pores are generally considered as stress concentrators and deteriorate the mechanical strength of material. By carefully controlling and tailoring the microstructure of the porous ceramic materials we can obtain the materials with improved properties.
Acknowledgements
This work was supported by the Next Generation Military Battery Research Center Program of the Defense Acquisition Program Administration and Agency for Defense Development.