Comparative Review of the Microstructural and Mechanical Properties of Ti-6Al-4V Fabricated via Wrought and Powder Metallurgy Processes
Article information
Abstract
This review examines the microstructural and mechanical properties of a Ti-6Al-4V alloy produced by wrought processing and powder metallurgy (PM), specifically laser powder bed fusion (LPBF) and hot isostatic pressing. Wrought methods, such as forging and rolling, create equiaxed alpha (α) and beta (β) grain structures with balanced properties, which are ideal for fatigue resistance. In contrast, PM methods, particularly LPBF, often yield a martensitic α′ structure with high microhardness, enabling complex geometries but requiring post-processing to improve its properties and reduce stress. The study evaluated the effects of processing parameters on grain size, phase distribution, and material characteristics, guiding the choice of fabrication techniques for optimizing Ti-6Al-4V performance in aerospace, biomedical, and automotive applications. The analysis emphasizes tailored processing to meet advanced engineering demands.
1. Introduction
Titanium alloys, particularly Ti-6Al-4V, have been identified as critical materials in high-performance applications such as aerospace, biomedical, and automotive industries due to their exceptional properties, including a high strength-to-weight ratio, excellent corrosion resistance, and biocompatibility [1-7]. However, the performance of Ti-6Al-4V alloy is highly dependent on its microstructure, which is significantly influenced by the processing parameters and methods employed during fabrication. The primary processing routes for Ti-6Al-4V are wrought processing and powder metallurgy (PM) routes, each with distinct mechanisms and outcomes [8–24]. Wrought processing involves mechanical deformation techniques like forging, rolling, and extrusion, followed by heat treatment steps to refine the microstructure and optimize the mechanical properties. Key parameters in this route include deformation temperature, deformation rate, and heat treatment conditions, all of which influence grain size, phase distribution, and the final mechanical properties of the alloy [10, 12, 17, 19, 25–33]. In contrast, powder metallurgy routes, such as LPBF and hot isostatic pressing (HIP), offer advantages in design flexibility and material utilization, especially for complex geometries and near-net-shape components [34, 35]. Critical parameters in LPBF include laser power, scan speed, layer thickness, and build environment, which affect melting, solidification rates, and residual stresses [9, 34]. HIP, a post-processing technique, uses high pressure and temperature to densify materials and eliminate porosity, with key parameters being pressure, temperature, and hold time, which influence porosity elimination and grain growth [24, 33]. While wrought processing provides components with balanced mechanical properties and good fatigue resistance, it can be limited in design complexity and material wastage. PM routes, particularly LPBF, enable the fabrication of intricate geometries with high material utilization but may require additional post-processing like HIP to enhance mechanical properties and reduce residual stresses [28]. This review aims to comprehensively compare the microstructures and mechanical properties of Ti-6Al-4V alloy produced through wrought and powder metallurgy routes, examining the influences of various processing parameters to elucidate the benefits and limitations of each method, thereby guiding the selection of appropriate processing techniques for specific applications. Understanding these parameters and their effects on microstructures and mechanical properties is crucial for optimizing the performance of Ti-6Al-4V alloy in its various applications.
2. Microstructure and Mechanical Properties of Wrought Ti-6Al-4V Alloy
The microstructure of wrought Ti-6Al-4V alloy is predominantly characterized by the presence of alpha (α) and beta (β) phases [31]. The specific wrought processing techniques employed significantly influence the distribution and morphology of these phases. Common processes such as forging and rolling typically result in a fine, equiaxed grain structure. For example, multidirectional isothermal forging (MDIF) achieves an ultrafine-grained microstructure with an average grain size of 0.14 μm, significantly enhancing the alloy's mechanical properties. Xu et al. [38] reported that the reduction in grain size due to MDIF leads to improve yield and tensile strengths. Similarly, rolling processes, whether at room temperature or under cryogenic conditions, increase dislocation densities and refine the grain structure, thereby enhancing hardness and strength. Yu et al. [39] observed that cryorolling at lower temperatures resulted in increased hardness values, reaching up to 383 HV. This increase is attributed to the refined grain structure as shown in Fig. 1, which increased dislocation densities, impeding dislocation motion and enhance the material's resistance to deformation. Heat treatment also plays a significant role in the microstructure of wrought Ti-6Al-4V alloy.
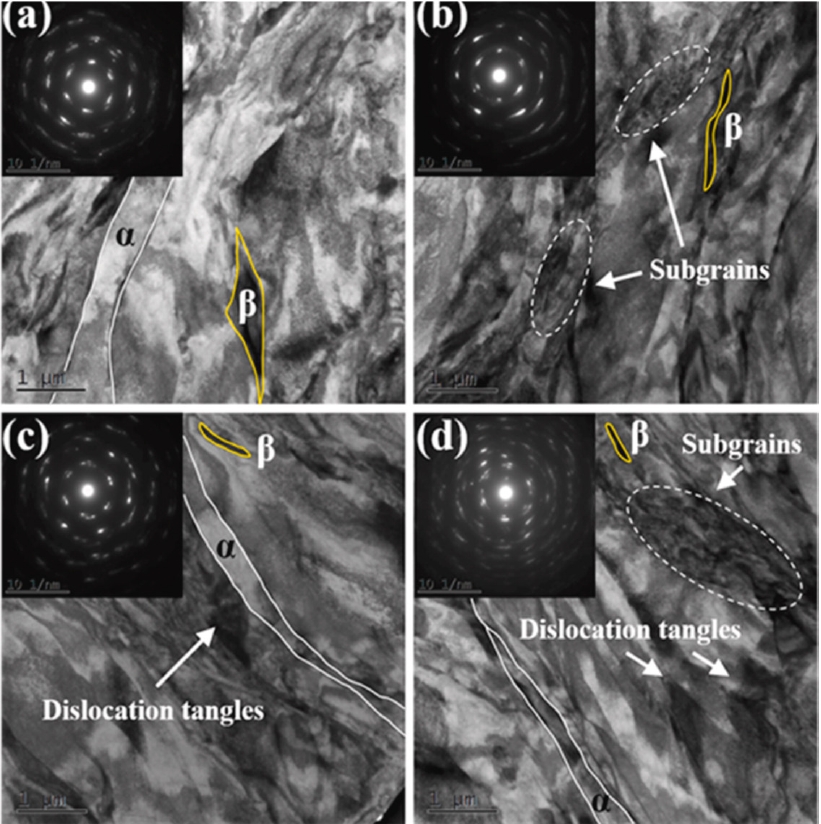
Fig. 1. Bright field transmission electron microscopy images of the specimens rolled at (a) 293 K-50%, (b) 173 K-50%, (c) 123 K-50%, and (d) 83 K-50%. Top left images show the selected area electron diffraction patterns of the corresponding specimens. This figure is reproduced from Yu et al. [39].
Fig. 1. Bright field transmission electron microscopy images of the specimens rolled at (a) 293 K-50%, (b) 173 K-50%, (c) 123 K-50%, and (d) 83 K-50%. Top left images show the selected area electron diffraction patterns of the corresponding specimens. This figure is reproduced from Yu et al. [39].
The high-temperature β phase undergoes transformation upon cooling, resulting in a mixture of equiaxed α and retained β phases [27]. The specific morphology and distribution of these phases depend on the thermomechanical processing parameters and the cooling rate. In wrought processing, deformation at high temperatures generally results in larger, more equiaxed α grains due to enhanced atomic mobility and grain growth during recrystallization. However, controlled deformation at lower temperatures can produce finer grains, which contribute to increased strength through grain boundary strengthening mechanisms [27]. The balance between these properties is critical, as overly large grains can compromise strength, while excessively fine grains may reduce ductility. The distribution of the α and β phases in wrought Ti-6Al-4V is crucial for its mechanical properties. Heat treatment, particularly solution treatment followed by aging, significantly influences this distribution. Solution treatment at temperatures within the β phase field dissolves alloying elements, creating a homogeneous single-phase β structure. Ahmed et al. subsequent aging promotes the precipitation of fine α particles within the β matrix, enhancing the alloy's strength through precipitation hardening [40]. The precise control of solution treatment and aging parameters is essential for optimizing the mechanical properties. Cyclic heat treatment, as discussed by Zhang et al. [41], breaks up the α lamellae to form a basket weave structure, which improves mechanical properties such as yield strength and ductility. This process enhances the alloy's ability to withstand mechanical stresses, making it more suitable for high-stress applications. The mechanical properties of wrought Ti-6Al-4V alloy, including strength, hardness, ductility, and toughness, are significantly influenced by the wrought processing techniques and subsequent heat treatments. Pratap et al. [42] reported higher tensile strength with increased thickness reduction from 25% to 75 % below the Tβ (β transus temperature) and higher impact strength in samples forged above or near the Tβ. These improvements are attributed to the refined microstructure and optimized distribution of α and β phases, which enhance the alloy's load-bearing capacity and resistance to deformation. Singh et. al (2023) [43] demonstrated that sub-transus annealing treatments enhance tensile strength and impact toughness while maintaining strength levels. These treatments involve heating the alloy to temperatures below the Tβ, promoting the formation of a fine, equiaxed α phase within a β matrix. This microstructural arrangement improves the alloy's strength and toughness by providing a balance between hard, load-bearing phases and softer, more ductile phases. The ductility and toughness of wrought Ti-6Al-4V alloy are also critical for its performance in various applications. Different microstructures influence these properties in distinct ways. For instance, Lei et al. [44] carried out the comparative study of mechanical properties for different morphologies of equiaxed (EM), bimodal (BM), fine lamellar (LM1) and coarse lamellar (LM2) microstructure of Ti64 alloy as shown in Fig. 2, obtained by controlling heat treatment temperature and cooling rate. They found that the bimodal microstructure exhibited the highest impact toughness due to its composite-like structure, which facilitates coordinated deformation. This microstructure consists of coarse primary α grains embedded in a fine α + β matrix, providing a balance between strength and ductility. The coarse α grains improve toughness by acting as crack deflection sites, while the fine α + β matrix enhances strength and hardness. In addition to strength and ductility, the hardness of wrought Ti-6Al-4V alloy is a critical property that determines its wear resistance and ability to withstand surface deformation. In summary, the wrought processing of Ti-6Al-4V alloy significantly influences its microstructure and mechanical properties. Techniques such as forging, rolling, and subsequent heat treatments refine the grain structure, enhance the distribution of α and β phases, and improve mechanical properties such as strength, hardness, ductility, and toughness. Optimized processing conditions, including proper heat treatment and thermomechanical processing, lead to improved performance, making the alloy suitable for demanding applications in aerospace, biomedical, and other high-performance fields.[6,13,14,40,45–48]
3. Microstructure and mechanical properties of Ti-6Al-4V alloy fabricated by powder metallurgy
LPBF and other AM techniques typically result in distinct microstructural characteristics due to their unique processing parameters. For instance, LPBF often produces a single-phase martensitic α' structure with high microhardness, as reported by Baghi et al. [49]. This fine lath morphology arises from the rapid cooling rates inherent to the LPBF process. In contrast, powder metallurgy (PM) parts display a two-phase α and β structure with comparatively lower microhardness. In a study by Long et al. [50] as shown in Fig. 3, ultrafine-grained Ti-6Al-4V alloys fabricated through high energy ball milling and spark plasma sintering demonstrated a remarkable 80-120% increase in compressive yield strength over coarse-grained alloys. This enhancement was due to grain refinement and strengthening from interstitial elements and oxide dispersion.
The significant microstructural differences between these methods underscore the substantial impact of fabrication techniques on phase composition and resultant material properties. The formation of a martensitic structure in LPBF parts can lead to increased hardness but reduced ductility. Shi et al. [51] noted that micro-hardness and tensile characteristics initially increased with laser line energy density (LLED) before declining, with optimal wear resistance achieved at an LLED of 0.24 J mm–1. Grain structure and refinement are critical to the mechanical performance of Ti-6Al-4V alloys produced by AM. LPBF frequently results in a columnar macrostructure of prior β grains with a mixed microstructure, leading to anisotropy in mechanical properties due to the preferred orientation of the α phase, as observed by Lei Meng et al. [52]. Conversely, techniques such as Electron Beam Melting (EBM) can produce equiaxed grains, which help reduce anisotropy. The study by Wang et al. [53] demonstrated that laser remelting could enhance microstructure by refining grain size, thereby increasing tensile strength by 12.7% transversely and 9.3% longitudinally. This process underscores the importance of grain refinement in enhancing both strength and ductility. Porosity control is another crucial factor in AM Ti-6Al-4V. Porosity can significantly impact mechanical properties, and techniques such as HIP are employed to mitigate this issue. Varela et al. [54] found that HIP treatment of LPBF parts resulted in a yield strength of 1.03 GPa and elongation of 16.7%, comparable to commercial Ti-6Al-4V products. The specific parameters used in AM processes, such as laser power and scanning speed, have a profound effect on the mechanical properties of Ti-6Al-4V. For instance, Zhao et al. [11] observed that increasing laser power refined the α′-lath thickness from 0.89 µm to 0.66 µm, thereby improving yield strength to approximately 1042.2 MPa. This refinement is crucial for enhancing the alloy's resistance to deformation. Singh et. al [43] demonstrated that sub-transus annealing treatments significantly improved tensile strength and impact toughness, making the material suitable for structural applications despite high oxygen content. Ductility and toughness are equally important for the performance of AM Ti-6Al-4V alloys. Lower HIP temperatures can retain a fine microstructure, resulting in higher elongation and better fatigue life, while higher HIP temperatures lead to a coarser microstructure with superior elongation and fatigue resistance, as shown by Ganor et al. [55].
It has also been reported that adding reinforcements such as TiC during the selective laser melting (SLM) process can significantly enhance wear resistance. Tang et al. [46] found that nanoscale TiC reinforcement improved the tribological properties of Ti-6Al-4V composites, achieving high relative density, hardness, and strength. These enhancements are attributed to the unique microstructure formed during the SLM process. Anisotropy in mechanical properties is a common challenge in AM Ti-6Al-4V alloys, influenced by build orientation and texture. Mian et al. [59] revealed significant anisotropy in elastic-plastic constitutive characteristics, with vertically built samples showing larger grain sizes and lower strength values compared to horizontally built samples. This anisotropy results from the columnar macrostructure and crystallographic texture developed during the AM process. Techniques such as laser remelting and careful control of thermal cycles during fabrication can help mitigate anisotropy by improving the uniformity of mechanical properties across different directions. Therefore, heat treatments play a crucial role in enhancing the mechanical properties of AM Ti-6Al-4V alloys. Singh et. al [43] found that solution treatment, aging and HIP treatments significantly improve impact toughness while maintaining strength levels. When comparing different AM routes, such as SLM and EBM, each method offers distinct advantages and challenges. SLM typically produces a finer microstructure with higher strength but may suffer from higher anisotropy and residual stresses. EBM, on the other hand, produces a more uniform microstructure with better ductility and reduced anisotropy.
Rashid et al. [58] found that EBM samples exhibited more uniform hardness and ductility compared to SLM samples, which showed higher strength and hardness but greater anisotropy. Surface roughness is another consideration, with SLM parts generally having higher surface roughness compared to EBM parts due to the finer powder used and higher energy input. Post-processing techniques such as HIP and surface machining are often required to improve surface finish and mechanical properties. Hybrid manufacturing approaches, which combine AM with traditional manufacturing techniques like forging and machining, offer the potential for enhanced mechanical properties and complex geometries. Ma et al. [17] studied the microstructure and mechanical properties of hybrid manufactured Ti-6Al-4V alloys, finding that the bonding zones exhibited higher tensile and yield strengths but lower elongation compared to purely additively manufactured parts. This approach leverages the strengths of both AM and traditional techniques to achieve superior performance. In biomedical applications, particularly dental prostheses, AM Ti-6Al-4V alloys exhibit promising properties. Yoshimitsu et al. [60] found that laser-sintered Ti-6Al-4V exhibits fatigue strength comparable to wrought alloys, with excellent corrosion resistance due to the formation of an oxide film on the surface. This makes AM Ti-6Al-4V suitable for long-term implantation and high-stress applications in the biomedical field. Overall, the microstructure and mechanical properties of Ti-6Al-4V alloy produced by LPBF and other AM routes are significantly influenced by processing parameters, post-processing treatments, and the specific AM technique used. Optimized processing conditions and advanced post-processing techniques, such as HIP and solution plus aging, are crucial for achieving superior mechanical properties. The addition of reinforcements and the use of hybrid manufacturing approaches further enhance the performance of AM Ti-6Al-4V alloys, making them suitable for a wide range of high-performance applications in aerospace, biomedical, and other industries.
The mechanical properties of Ti-6Al-4V alloys produced through various processing routes are summarized in Table 1, with a visual representation provided in Fig. 4. These routes include both traditional wrought methods and advanced powder metallurgy (PM) techniques. Each processing method, along with its corresponding heat treatments, has a notable influence on key material characteristics such as grain size, yield strength, tensile strength, and elongation. Wrought methods, such as forging and rolling, typically result in fine equiaxed or sub-micron grain structures. These processes benefit from additional heat treatments like mill annealing or cryorolling, which help refine the grain size and improve overall mechanical performance. The cryorolling process, for instance, is conducted at low temperatures, which leads to sub-micron grain structures and results in slightly reduced tensile strength and elongation, but it still maintains competitive yield strength. The PM techniques—such as HIP, LPBF, and EBM—also produce fine or refined grain structures, which enhance the material’s mechanical properties. In particular, the as-built martensitic structure created by LPBF offers a higher yield and tensile strength than traditional wrought methods. However, the elongation may be reduced unless the material undergoes post-processing, such as HIP. This post-treatment step is essential for reducing porosity, refining the grain size further, and ultimately improving the ductility of the alloy. Additionally, solution treatment and aging at elevated temperatures (e.g., 950°C) are commonly applied to some PM routes to further refine grain structure and enhance the material’s mechanical properties, achieving a balance between strength and ductility. These treatments, combined with advanced PM methods, demonstrate the versatility of Ti-6Al-4V alloys and the significant impact of processing routes on their overall performance.
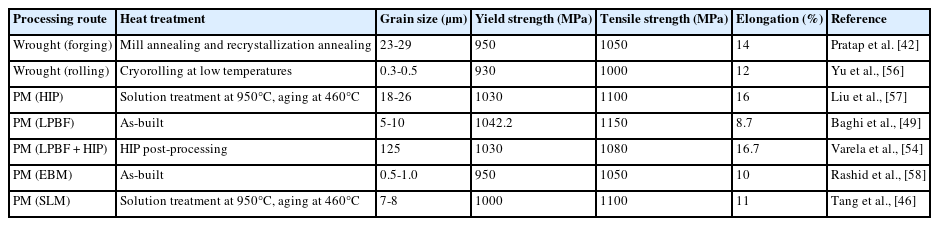
Comparison of yield strength, tensile strength and elongation of Ti-6Al-4V alloys fabricated through different processes
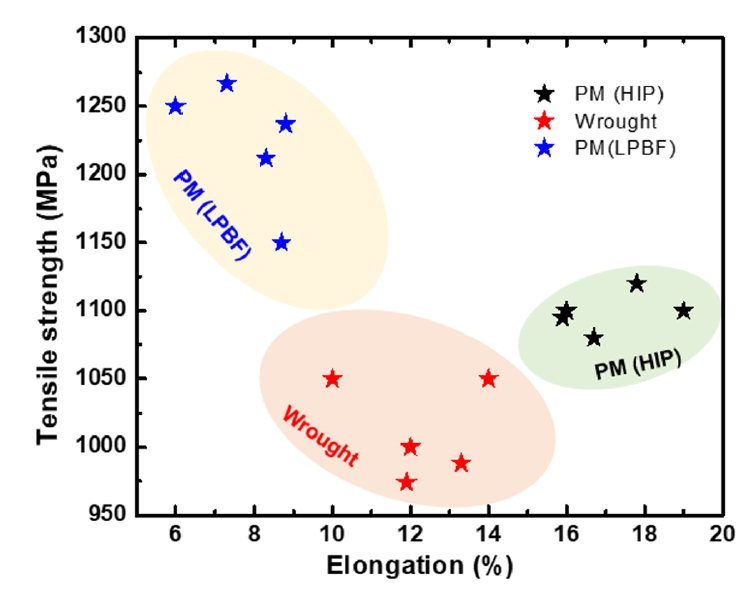
Comparison of tensile strength and elongation of Ti-6Al-4V alloys fabricated trough different processes.
A key strategy to achieve a balance between tensile strength and elongation is the careful optimization of heat treatment parameters. Solution treatment followed by aging helps refine the microstructure by optimizing the distribution of α and β phases, which enhances tensile strength while preserving ductility. In addition, post-processing techniques like HIP play a crucial role in reducing porosity, refining the microstructure, and relieving residual stresses, leading to simultaneous improvements in both strength and elongation. Moreover, hybrid manufacturing techniques that integrate additive manufacturing with traditional methods offer enhanced control over microstructural characteristics and mechanical properties, providing a means to improve both properties simultaneously. By fine-tuning processing methods and tailoring microstructures, the trade-off between tensile strength and elongation can be minimized, leading to superior performance in advanced engineering applications.
4. Summary
1. Ti-6Al-4V's microstructure is highly dependent on the fabrication method. Wrought processing yields a fine, equiaxed α-β grain structure, enhancing strength, ductility, and toughness. In contrast, PM techniques like LPBF result in a martensitic α′ structure with high microhardness but lower ductility.
2. Processing methods and subsequent treatments significantly impact mechanical properties. Wrought Ti-6Al-4V offers balanced mechanical properties and good fatigue resistance, whereas PM methods, though enabling complex geometries and high material utilization, require post-processing like HIP to improve properties and reduce residual stresses.
3. Wrought processing is limited in design complexity and has higher material wastage. PM routes provide superior design flexibility and efficient material use but depend on extensive post-processing to achieve desired mechanical properties.
4. Selection of fabrication methods should align with application requirements. PM techniques are advantageous for intricate geometries and minimal material waste, while wrought processing is preferable for robust mechanical properties with less need for post-processing.
5. Both methods have optimization potential. Advances in controlled thermomechanical treatments for wrought processing and improved parameter control in LPBF can enhance Ti-6Al-4V properties. Hybrid manufacturing approaches, combining strengths of both methods, could benefit applications needing complex geometries and high-performance properties.
Notes
Conflict of Interest Declaration
JH. Kim serves as an editor of the Science editing, but has no role in the decision to publish this article. Except for that, no potential conflict of interest relevant to this article was reported.
Author Information and Contribution
Raj Narayan Hajra: Ph.D. researcher, data collection, analysis, writing a manuscript; Gargi Roy : Researcher, data collection, analysis; An Seong Min: Undergraduate student, data collection; Hyunseok Lee: Principal Researcher, design of concept and supervision; Jeoung Han Kim: Professor, design of concept and supervision.
Acknowledgement
This research received support from two distinct projects under the Technology Innovation Program. The first project (grant number 20016092) focused on the development of a Ti–6Al–4V alloy plate with a thickness of 100 mm, tailored to meet aerospace material specifications. The second project (grant number 20010047) aimed at the development of a deoxidation refining process. This process was designed for handling over 100 kg per day of off-grade Ti scrap, facilitating the production of 4N5 grade ingot, and utilizing powder technology. Both projects were funded by the Ministry of Trade, Industry &; Energy (MOTIE), Korea.